With the growth of ‘white biotechnology,’ industrial fermentation processes and large-scale fermenters will play a key role. Presented here are some design considerations
In fermentation, microorganisms, animal and plant cells are used to produce chemical compounds. Enzymes, pharmaceutical ingredients, amino acids or vitamins, as well as various monomers are manufactured on the basis of renewable resources as metabolic products. Bioprocesses based on fermentation are becoming more and more popular in industry, not only because of their environmental friendliness and energy efficiency, but also because they can be carried out in very simple and efficient bioreactor systems.
The necessity for industrial chemistry to move to energy-efficient processes and renewable, bio-based products induces a lively development activity in the field of industrial fermentation. In recent years, besides the classical aerated fermenters there are also new fermenter concepts coming into play.
Examples for such modern fermenter concepts are the following:
- Power-to-food fermentation, which involves microorganisms that synthesize proteins only from gases like CO2, O2 and H2 used in the manufacture of plant-based meat, for example
- Power-to-gas fermentation, involving microorganisms that, for example, produce methane from H2 and CO2 in a very energy efficient way — a quite promising method for energy storage.
The development of new processes always requires a safe scaleup from laboratory to production scale [1]. Today, fermenters in world-scale plants reach sizes of several hundred cubic meters, and for low-viscosity processes based on bacteria, of more than 800 m3. However, fermenter sizes of considerably more than 1,000 m 3 are being examined in feasibility studies.
For these new fermentation processes, it is therefore necessary to examine to what extent the existing know-how, for example, for stirred, aerated fermenters or hydrogenation reactors, can be used for a safe scale-up and which new requirements have to be considered.
Aerated fermenter design principles
Many aerobic-fermentation processes are operated very efficiently in stirred, aerated fermenters. In general, the requirement for a fermenter is that it provides conditions for a high productivity of the microorganisms. This includes the optimal concentration of the components required for the metabolism, such as dissolved O2 or various nutrients and substrates. To achieve this, added air as the O2 source must be dispersed, and additives, such as nutrients and pH-regulating substances, must be efficiently homogenized. Another crucial requirement is a constant and homogeneous temperature field throughout the entire fermenter. Among numerous energy sources and sinks, the metabolic heat, together with the energy dissipated by the agitator, are usually the dominant energy sources to take into account in the energy balance.
The fermenter design and scale-up is based on the target productivity, which decisively correlates for a given bioprocess with the volumetric oxygen uptake rate (OUR), which varies with batch time. To comply with the mass balance, the volumetric air feed rate, vvm, is usually kept constant during scaleup, as shown in Equation (1):
with the normalized gassing rate, ˙q N, the liquid phase fermentation broth volume V and the internal fermenter diameter d1. Under these conditions, scaling up with geometric similarity results in a linear increase of the superficial gas velocity, vsg, with the vessel diameter, d1, as
where A is the cross-sectional area of the fermenter. The volumetric oxygen transfer rate, OTR, through the gas-liquid Interface is determined from Equation (3):
OTR must satisfy the volumetric oxygen uptake rate OUR of the microorganisms. The difference between the saturation concentration, c*, and the liquid bulk concentration, cL, represents the driving force for gas-liquid mass transfer. The gas-liquid mass-transfer capacity for agitated processes, kLa, can be correlated by Equation (4):
where k, α and β vary over a wide range depending on the gas-feed device, impeller type and material properties, such as the coalescence behavior of the gas-liquid system [2]. Following the above-mentioned scale-up rule and target, the power-to-volume ratio, P/V, can clearly be decreased with increasing scale. This is first of all because vsg increases with scale (~ d1) and also since the average hydrostatic pressure in the larger tanks operated at higher liquid level results in an increased saturation concentration, c*, and therefore driving force c*– cL.
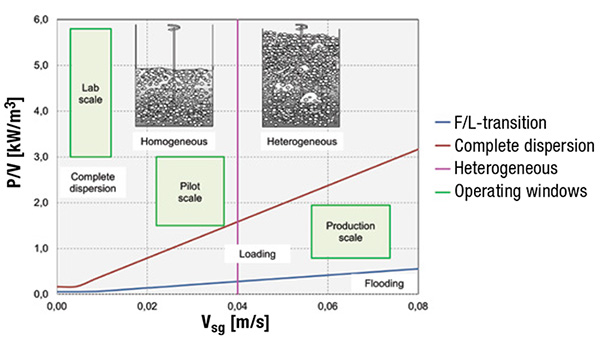
FIGURE 1. This flow map of agitated fermenters shows a transition from the homogeneous-flow regime and complete dispersion condition in laboratory and pilot scale, to an operation in the heterogeneous-flow regime and loading condition in production scale
This leads to operation windows, according to Figure 1, with reduced specific agitator power and higher gas velocities going from laboratory through pilot to production scale. This trend can lead to a drastic change in hydrodynamics. Figure 1 shows a typical scenario with a transition from the homogeneous-flow regime and complete dispersion condition in laboratory and pilot scale to an operation in the heterogeneous-flow regime and loading condition in production scale. This means that the bubble-size distribution is narrow, and the bubbles are rather evenly dispersed in the small-scale fermenter. In production scale, large bubbles appear, and an increasingly inhomogeneous local distribution of the gas phase can occur. Finally, flooding must be strictly avoided, as gas-liquid mass transfer would drastically break down. The flooding limit can be shifted towards higher gas flowrates when using modern concave-type impellers instead of the traditional ones, such as, for example, flat blade disc (Rushton) turbines or pitched-blade turbines. The effect of the interaction of the impeller type with, for example, the feeding device or internal heat exchangers and the influence of the operation range on the agitator system performance can be investigated with model fluids in sufficiently large scale of ~1 m3 as shown in Figure 2. This allows a comparison of agitator and fermenter systems regarding the general flow pattern with possible stagnant areas, gas-liquid mass transfer and blend time.
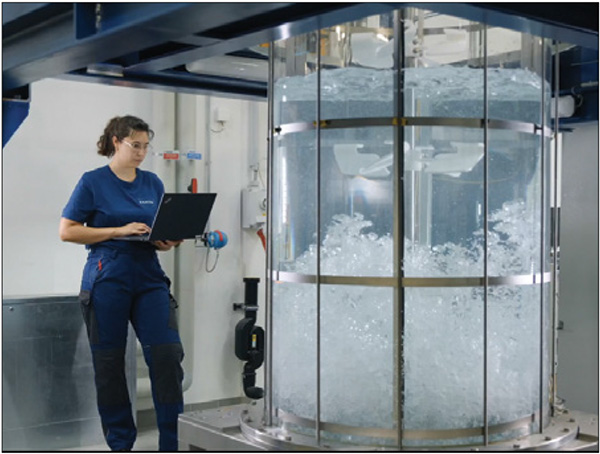
FIGURE 2. This 2-m3 fermenter model is used for research and development with model fluids
Process design for pure gases
Fermentations with pure gases have recently become increasingly important. Examples are power-to-food or power-to-gas processes. These processes have both similarities to traditional aerobic fermentations and significant differences that are more reminiscent of pure gas reactions such as hydrogenation. Many of these new processes have so far been operated on a pilot scale or smaller production scales. To benefit from “economies of scale,” the understanding of these processes still needs to be significantly improved. However, the experience already gained with aerobic fermentation and reactions with pure gases can be helpful. Reactors are therefore used for pure gas reactions, using gas recirculation from the headspace. This variant is particularly suitable for processes in which the gas supplied to the process needs to be completely converted. The fresh gas is usually introduced into the bottom of the reactor and split into small gas bubbles by a primary disperser. At the same time, unreacted gas is redispersed from the headspace via a hollow shaft using a self-inducing impeller type (see upper impeller in Figure 6).
An assessment of the fermenter performance can be defined analogous to the OTR in aerobic fermentation for a suitable gas component (educt or product)
where GTR is the volumetric gas transfer rate, qGc,i, the volumetric flowrate of the gas component irelative to the input (in) and output (out) of the fermenter and VL the volume of liquid in the fermenter. To optimize the GTR, on the technical side, the mass transfer of the supplied gas components from the gas to the liquid phase, which is necessary for product formation, must be maximized. On the biological side, suitable microorganisms must be used or developed that enable the highest possible absorption and conversion of the gas components into the product.
As for aerobic fermentations the volumetric gas transfer rate can be described by Equation (6):
The term c*( Gc i) is the saturation concentration of gas component i, and cL (Gci) the liquid bulk concentration of gas component i. The gas-liquid mass transfer capacity, kL a can be determined from Equation (4) and is hence affected by the same parameters.
The main limitation of GTR could be the very low solubility of a gas component used in the aqueous fermentation broth. Hydrogen, for example, has a solubility that is over 100 times lower than for carbon dioxide.
Mixing concept and scale-up
When scaling up a fermentation process, the goal is often to keep the volumetric transfer rate of the gaseous components, and thus also the volumetric uptake rate and conversion of the gas component by the microorganisms, at least constant. To achieve this, the concentration of the biomass and the gassing rate per volume of fermentation broth, vvm, are kept constant. As described in Equation (2), this prerequisite results in an increased superficial gas velocity, vsg.
Typically, the main mixing task is to efficiently disperse the added gas to ensure a sufficient gas/liquid mass-transfer interfacial area. This is a function of the specific power input and, equally important, the impeller and geometric fermenter setup.
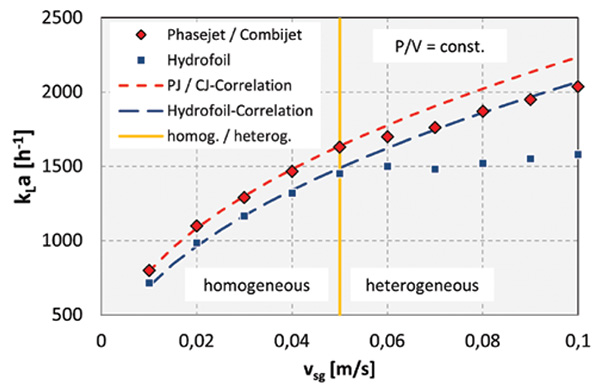
FIGURE 3. The impact of heterogeneous-flow conditions on the kLa value can be seen here
One important process parameter to be considered while scaling up is the transition from homogeneous to heterogeneous flow (upper magenta line in Figure 1), which takes place in pure water at vsg ~0.05 ms–1. In non-coalescing liquids, the transition can occur at significantly lower vsg [3]. The kL a correlation found in the homogeneous regime cannot be extrapolated to the heterogeneous regime. When passing the transition from homogeneous to heterogeneous flow, the kLa correlation may change drastically or even break down completely for some impeller systems (Figure 3). In order to carry out a safe scaleup, the kLa correlation used for scaleup should ideally be based on data gained with the real gas-liquid system and the respective operating conditions in terms of P/V and vsg, expected in production scale. In case of an operating point in the heterogeneous flow regime, the impeller choice can also become crucial (see Figure 4). If it is not possible to carry out small-scale tests with the real gas-liquid system, it is advised to find a representative model system.
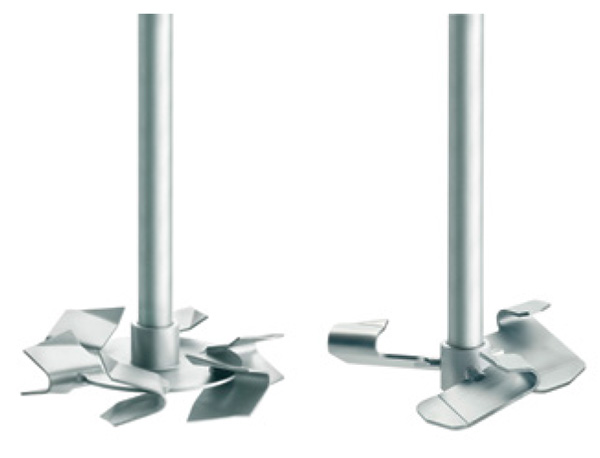
FIGURE 4. Shown here are two high-performance impellers for fermentation processes (left: Phasejet, right: Combijet)
In aerobic fermentations, vessels with a large height-to-diameter ratio are commonly used. Fresh gas is supplied and dispersed in the bottom area by an impeller working as a primary disperser. Additional impeller stages ensure an intensive redispersion of the gas over the fermenter height, thus promoting the gas-liquid mass transfer (Figure 5). When it comes to the geometric design of aerobic fermenters, economic, process- or design-engineering reasons, among others, are weighed against each other, since there is no common optimum.
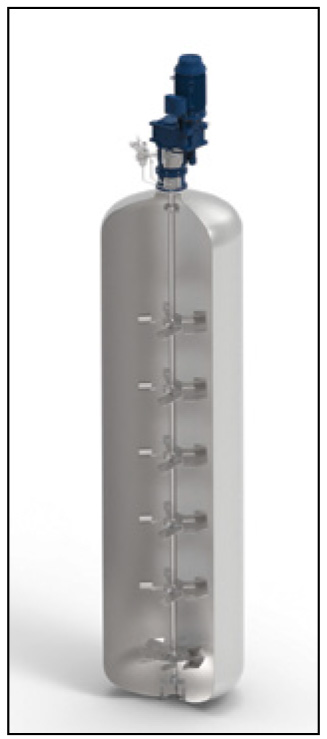
FIGURE 5. This cutaway shows a concept for a large industrial stirred and aerated fermenter
For fermenters that are operated with pure gases, the process requirements basically result in two possible reactor alternatives. On the one hand, analogous to aerobic fermentations, a reactor with a high aspect ratio (Figure 5) would be advantageous. In addition to the positive influence on the saturation concentration and the kLa, this will also prevent a short-circuit between the inlet and outlet flows. On the other hand, gas from the headspace could be returned to the fermentation broth using the combined gassing system commonly applied for reactions with pure gases. This setup includes a primary gas disperser in the bottom and a self-inducing gassing impeller, as in the commercial unit connected to a hollow shaft shown in Figure 6. In this way, the kLa would be increased by an increasing gassing rate using the recirculated gas from the headspace. This type has been successfully applied for hydrogenation processes for many years.
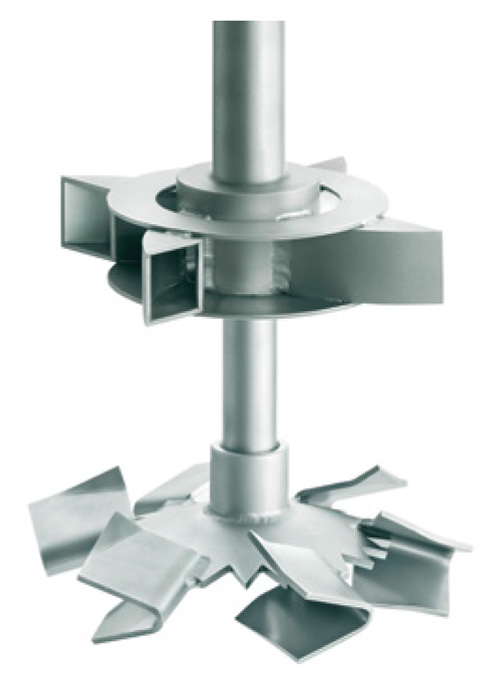
FIGURE 6. This “combined gassing” concept can be applied for “pure gas” fermenters
During scale-up, it must be checked whether the increasing gassing rate can still be dispersed by the primary disperser (lower impeller). If this is not the case and the primary disperser is flooded, this will have a significant negative impact on the mass transfer (kLa). The larger the aspect ratio of the vessel, the closer the operating point to the flooding curve.
If there is a significant difference between the inlet and outlet gassing rates due to the metabolic conversion, it must also be checked whether there are significant gradients in the superficial gas velocities in the fermenter. This would also result in significant kLa and GTR gradients, which must be considered in the process design and geometric optimization of the fermenter.
Apart from the challenges from the process engineering point of view, the ongoing increase in reactor sizes also bears huge challenges from the mechanical point of view.
Agitator components
The following three key factors have to be considered when designing agitator components for fermentation applications: cleanability, cost and flexibility.
Agitators must be designed to allow for thorough cleaning to prevent contamination of subsequent batches. Cleanability can be improved by using smooth surfaces, avoiding tight spaces, and using materials that are resistant to corrosion and chemical attack.
Agitator components must be cost-effective to manufacture and to install. This may require using less expensive materials or simpler designs.
Agitators must be able to be disassembled and reassembled easily for maintenance or repair. This may require using components that are not welded together.
As a consequence, there are three main approaches to design agitator components for fermentation applications, as follows:
- Gaskets are used to seal connections between components. This approach is relatively inexpensive and flexible, but cleanability might be more complicated compared to alternative approaches
- Welding components together creates a continuous, sealed surface. This is the best cleanable solution, but the least flexible
- Special solutions can be used to combine the advantages of gaskets and weldings. For example, components can be welded together with a smooth, flush surface
In addition to the design of the agitator components themselves, there are several guidelines to consider when designing the interior of a fermenter, including the following:
- Horizontal surfaces should be avoided as they might trap product residue, leading to contamination
- Surface quality is important. A polished surface is easier to clean than a rough surface
Mechanical design principles
Aerated fermenters tend to have a very low natural-vibration frequency. Main reasons for this are the usually quite large height-to-diameter ratio of the apparatus in combination with very thin vessel walls, as operating and design pressures are usually quite low.
When operating an aerated fermenter, the frequencies created by agitation run the risk of matching the natural frequency of the vessel, the agitator, or the vessel-agitator system. This can lead to strong vibration or even serious damage of the system.
To identify the natural frequency of the vessel-agitator system, it is necessary to gather information from both the agitator manufacturer and the vessel manufacturer. This can be a complex and time-intensive process.
To visualize the interaction of frequencies and agitator speed, a so-called Campbell diagram (Figure 7) can be used. This diagram shows all the excitation frequencies and natural frequencies. It can be used to identify potential resonance risks.
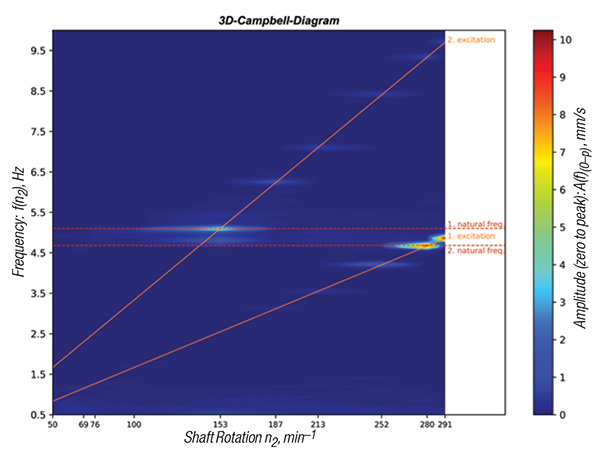
FIGURE 7. The Campbell diagram can help visualize the interaction of vibration frequencies and agitator speed, which enables the identification of potential resonance risks
To take different filling levels into account, it is recommended to perform a fluid-structure interaction (FSI) calculation. This calculation uses finite element analysis to model the interaction of the fluid and the structure.
With this information, it is possible to check the agitation frequencies against the natural frequencies of the vessel-agitator system. If there is no match, the operation of the system is non-critical. If there is a match, design changes are required.
Final remarks
Industrial fermentation is becoming more and more important in the chemical process industries. The main reason for this: as a bio-process, it is “copied from nature” and therefore an energy-efficient alternative to produce a lot of important chemicals and intermediates — in direct competition to the classical chemical synthesis. Therefore fermentation helps to provide more energy efficient production routes with a much better ecological footprint.
The better the fermentation process is understood — and the earlier the significant process parameters are identified — the quicker and smoother the development and scaleup of an industrial fermentation will proceed.
Economies of scale are crucial for industrial fermentation because they allow for higher production volumes, lowering the cost per unit of the fermented product. This reduction in cost enables companies to offer competitive pricing in the market, expanding their customer base and increasing profitability.
To finally engineer and build a large world-scale fermenter, the focus needs to be expanded to mechanical robustness. Besides requirements like cleanability and flexibility the fermenter needs to be set up in a way to avoid vibration or resonance risks.
Edited by Gerald Ondrey
References
1. Gezork,K. and Rosellen, M., Auslegungssache – Scale-up und Engineering von Fermentern, CITplus, Vol. 20, January-February, pp. 2-4, 2017.
2. Gezork, K., Bujaski, W., Cooke, M. and Nienow, A., Mass transfer and hold-up characteristics in gassed, stirred vessels at intensified operating conditions, Chem. Eng. Res. Des., Vol. 79, pp. 965–972, 2001.
3. Gezork, K., Bujaski, W. Cooke, M. and Nienow, A., The transition from homogeneous to heterogeneous flow in a gassed, stirred vessel, Chem. Eng. Res. Des., Vol. 78, pp. 363–370, 2000.
All figures courtesy of Ekato RMT.
Authors
Wolfgang Keller is head of R&D at Ekato Rühr- und Mischtechnik (RMT) GmbH (Hohe-Flum Str. 37, 79650 Schopfheim, Germany; Phone +49-7622-29-468; Email: [email protected]). He has more than 20 years of professional experience in process engineering and development, especially focused on polymers and minerals processing applications. Keller holds a master’s degree in chemical process engineering from the University of Karlsruhe (Germany).
Klaus Gezork is senior project engineer in Ekato RMT’s R&D Dept. (same address as above, Phone +49-7622-29-387; Email: [email protected]. He has more than 20 years of professional experience in process engineering and development, especially focused on fermentation and high viscous mixing applications. Gezork holds a master’s degree in process engineering from the Technical University of Aachen (Germany) and a Ph.D. on gas/liquid mixing from the University of Birmingham (U.K.).
Bernd Nienhaus is business development manager at Ekato RMT (same address as above; Phone +49-7622-29-276; Email: [email protected]). He has more than 20 years of professional experience in process development and optimization with main focus on mixing in crystallization and polymerization processes. Nienhaus holds a Ph.D. in industrial chemistry from the University of Oldenburg (Germany).
Niclas Popp is head of marketing at Ekato RMT (same address as above; Phone +49-7622-29-522; Email: [email protected]). He has more than 10 years of professional experience in the pharmaceutical and chemical industry with a strong focus in fermentation projects in recent years. Popp holds a master’s degree in industrial engineering and management from the Baden-Württemberg Cooperative State University in Heilbronn, Germany.